Explaining Helion’s fusion fuel: D-He-3
Dr. David Kirtley, CEO
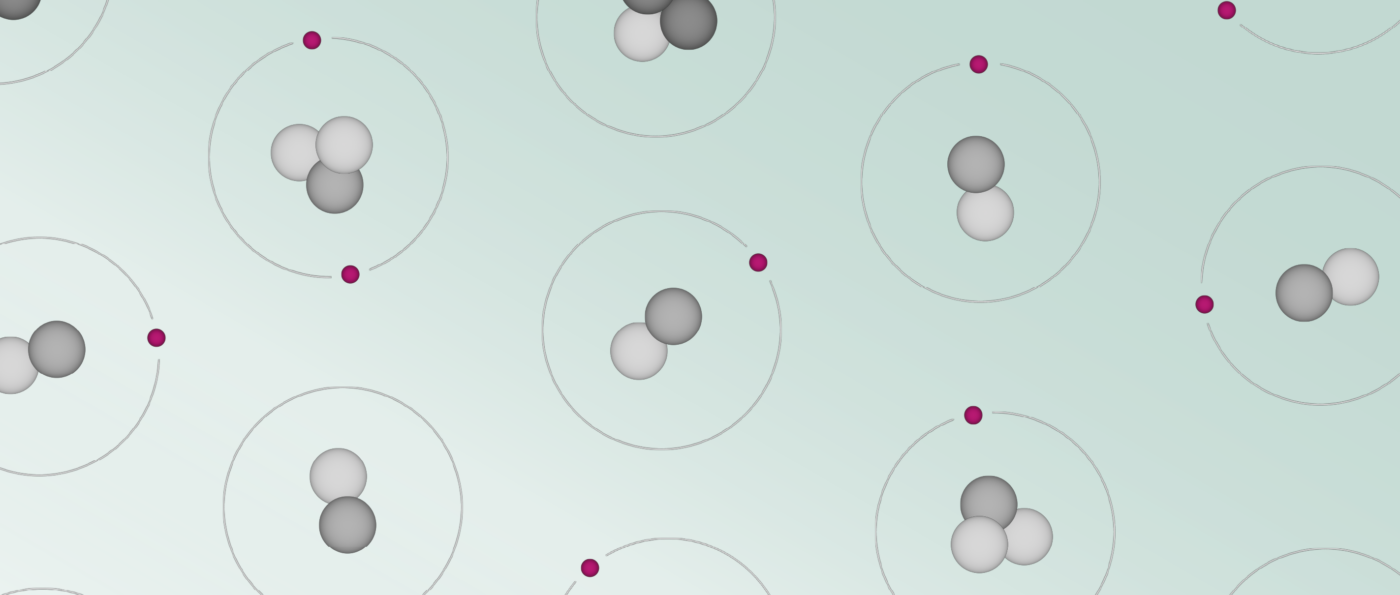
We use a unique fusion fuel: Deuterium-Helium-3 (D-He-3). This sets us apart from most fusion approaches because it requires higher temperatures than more traditional fuels while relying on a fuel source that isn’t naturally abundant on Earth.
Why did we choose this approach?
Among other benefits, D-He-3 maximizes our ability to directly capture electricity, a large advantage when building a fusion system for commercial deployment. To explain, it’s easiest if we start with the properties of the single atom.
Helion has used, or will use, three fuels at some stage in our path to commercialization: deuterium, tritium, and helium-3. In their gaseous forms, each can be heated to plasma conditions and used in a fusion reaction. Helion’s machines inject these fuels as a gas and heat them to plasma conditions. We then use magnets to accelerate, merge, and compress these plasmas until fusion occurs. Depending on the injected fuel mixture, varying results and byproducts occur.
Deuterium
Deuterium is an isotope of hydrogen, the universe’s lightest and most abundant element. Unlike ordinary hydrogen, deuterium has a neutron that makes it slightly heavier without changing its chemical properties. Deuterium is stable and occurs in about one of every 6,000 hydrogen atoms, making it naturally abundant.
Tritium
Tritium is another hydrogen isotope, but it has two neutrons and is unstable, with a half-life of 12.3 years, decaying into helium-3. Tritium is also extremely rare in nature, so it is often produced by destroying lithium with neutrons in a process known as breeding.
Helium-3
Helium-3 is an isotope of helium, the second lightest element on the periodic table. Unlike regular helium, which has two neutrons, helium-3 has only one, making it lighter. Although helium-3 is stable and naturally occurring in the universe, it is rare on Earth because it tends to escape our atmosphere. We have created helium-3 through the fusion of deuterium with deuterium, a process we’ll discuss further.
With these fusion fuels, there are three fusion reactions most likely to occur when the right conditions are met: deuterium-deuterium (D-D), deuterium-tritium (D-T), and deuterium-helium-3 (D-He-3). While other fusion reactions are technically possible, they are highly impractical.
Deuterium-Deuterium (D-D)
D-D fusion requires temperatures around 100 million degrees Celsius (M°C). This reaction yields a 1 MeV* triton (tritium nucleus) and a 3.02 MeV proton (hydrogen nucleus) in 50% of reactions, while the other 50% results in a 0.8 MeV helion (helium-3 nucleus) and a 2.45 MeV neutron. Despite being readily available, D-D’s energy output isn’t high enough to compensate for the energy needed to initiate the reaction. For a commercial system, D-D likely cannot be a working solution.
Deuterium-Tritium (D-T)
D-T fusion starts to occur at around 50 M°C, producing a 3.5 MeV alpha (helium-4 nucleus) and a 14.1 MeV neutron. While it requires lower temperatures than the other two reactions, the energetic neutrons it produces pose significant challenges for commercial operations, most notably material degradation. Moreover, the energy created from neutrons in a D-T reaction is not charged, so it can only be captured through thermal conversion. The neutrons are captured using liquid lithium blankets, which require the reaction to permanently consume rare lithium. The need for a lithium fuel, in addition to replacing degraded materials regularly and accounting for the construction of large cooling towers drives up capital costs, making the electricity created through the process more expensive.
Deuterium-Helium-3 (D-He-3)
D-He-3 fusion requires the highest temperatures, about 200 M°C, which does pose an engineering challenge due to the need for stronger magnets and putting more energy into the system. However, both challenges can be addressed through designing better magnets or more efficient circuits. This reaction produces a 3.6 MeV alpha and a 14.7 MeV proton. However, with a D-He-3 mixture, D-D reactions can still occur, resulting in five possible outputs: 3.6 MeV alphas, 0.8 MeV helions, 14.7 MeV protons, 1 MeV tritons, and 2.45 MeV neutrons.
Despite the higher temperature requirements, some key advantages of D-He-3 begin to emerge. The most significant is the type of particle it emits. Protons, unlike neutrons, can be contained using magnetic fields, reducing the material wear and shielding requirements compared to a D-T reaction. D-He-3’s reactions yield substantial energy – 18.3 MeV – more than tritium, and without the higher-energy neutrons. This is a significant benefit in Helion’s systems, as we directly recover electricity from this process. By not relying on a thermal conversion, we do not need to build large cooling towers and steam turbines; instead, we can pull energy directly from the reaction. For a commercial system, this helps to increase efficiency and reduce capital and operating costs.
And while helium-3 isn’t abundant on Earth, it can be created through a process of breeding within the D-D reactions. Additionally, the tritium created through the process decays into helium-3, which we can use in our fusion process.
From testing to commercialization
While we run most tests with D-D due to its low cost and relative availability, we would not choose this as a commercial fusion power plant fuel because of its limited energy output. The energy created from a D-D reaction just cannot outproduce the energy required for the reaction in the first place.
D-T fuel allows us to achieve fusion conditions at lower temperatures, which is useful for testing our machines. However, the challenges associated with neutron production make it impractical and expensive for our long-term commercial use.
That’s why we chose D-He-3. Although it requires higher operational temperatures, the benefits of reduced neutron output and efficient energy production make it the optimal choice for Helion’s commercial fusion power plants. Our approach, leveraging Field Reversed Configurations (FRCs), is particularly suited to achieve these high temperatures, making D-He-3 fusion feasible.
By focusing on D-He-3 fusion, we believe we are on a more efficient and cost-effective path to commercial fusion power. Our first-principles approach, assessing each fuel’s potential for commercialization, consistently pointed to D-He-3 as the fastest route to market-ready fusion energy. While there are intermediary challenges, overcoming them promises the most robust and economically viable solution for fusion power.
* eV (electron Volts) is a unit of temperature; it refers to the speed at which the particle travels. The higher the number, the faster – or more energetic – it is. More energetic particles = higher temperature = more energy released.